Self-healing materials are a class of smart materials capable of autonomic repair after damage at ambient temperature without external intervention. 1,2 Since White et al reported the first example of self-healing polymeric material capable of autonomic repair without any external intervention, 3 the concept of self-healing functionality for materials has captured the imagination of academia and industry alike, and spawned the development of various chemistries and concepts for designing self-healing functionality into polymeric materials. These chemistries and concepts include autonomic options that do not require any external intervention, 4-6 and non-autonomic options that might require heat or ultraviolet radiation, for example, to facilitate the healing response. 7,8 Few applications have the potential to benefit more from the life extension gained by incorporating self-healing functionality into a protective coating system than offshore oil and gas structures that face harsh corrosive environments paired with costly maintenance and downtime.
Chevron, a major oil and gas asset owner, sought out a solution to one of the two most common failure mechanisms affecting protective coatings: micro-cracking and the propagation of micro-cracks that lead to water ingress to the substrate and subsequent corrosion. This was accomplished by conducting a research study in collaboration with Rust-Oleum, a manufacturer of a range of protection solutions for metal substrates, and Autonomic Materials, a supplier of microencapsulated healing agents. The primary goal of the study was to develop, validate and deploy a self-healing coating system that would extend the life of standard protective coatings, resulting in reduced costs and labor associated with coating maintenance for off-shore applications. The resulting coating systems were tested at a third-party laboratory, Charter Coating Services (2000), Ltd., in Calgary, Alberta.
The service life of a protective coating can be greatly increased if damage is arrested at the onset of cracking and micro-cracking. Incorporating self-healing functionality into the coating allows the arrest and retardation of mechanical damage, resulting in prevention of corrosion undercreep and delamination at the metal-coating interface. This allows the bulk coating to adhere to the substrate for a longer period of time, thereby reducing the need for maintenance and subsequent labor costs over the life of the asset.
Self-healing functionality in protective coatings has the greatest potential benefit in applications for which inspection and maintenance are difficult due to the location of the asset, and where downtime is costly. Off-shore environments represent some of the most severe corrosion conditions faced by any industry. Compounding these corrosive environments, the increased cost of labor offshore, and the cost of downtime when sections of the platform are taken off-line for maintenance, off-shore oil and gas structures exemplify the types of assets that benefit most from protective coatings that incorporate self-healing functionality. Based on data reported in a NACE International Corrosion 2016 paper, 9 we estimate the cost of paint to be ~5% of all painting activities for an off-shore oil and gas platform. As such, extending the service life of protective coatings could result in significant cost savings due to a decrease in the frequency of maintenance. Although the associated labor costs are less significant, benefits from this technology also extend to on-shore facilities.
Optimizing Maintenance Costs
As a major oil and gas asset operator, Chevron is acutely aware of the total cost of corrosion to their operations and is actively engaged in a range of projects aimed at evaluating and ultimately deploying new technologies in an effort to minimize the total costs associated with coating maintenance. These costs include surface preparation, application of the coating systems, lost productivity due to asset downtime, and cost of bringing maintenance personnel to the job site; which, especially offshore, can have both cost and safety implications.
This article presents the outcome of the first phase of a multi-phase collaboration between Chevron, Rust-Oleum and Autonomic Materials. The objective of the first phase of this study was to characterize the self-healing mechanism and performance of METAPrime, a commercially available self-healing coating. In this phase, small-scale damage was imparted into the coating system in an effort to simulate micro cracking, which typically renders coating systems susceptible to moisture ingress. Moisture ingress is known to compromise adhesion of a coating to the underlying substrate and cause corrosion undercutting (creep), resulting in the need for maintenance.
Healing Mechanism
The self-healing additive is comprised of a liquid healing agent that incorporates polymer precursors based on epoxy, siloxane and/or alkyd chemistries, encapsulated in microcapsules measuring 10-20 microns in diameter made of a polymeric shell wall. After manufacturing in an oil-in-water emulsion, the microcapsules are isolated via spray drying. The microcapsules are then incorporated into a coating formulation with other dry pigments and other particulate additives. The mechanism of restoring the coating’s protective capability depends on the type and scope of the damage. Micro-cracks (Figure 1a) are plugged by the polymerized healing agent while the edges of larger-scale damage (Fig1b) and cracks stemming from them are sealed. Figures 2 and 3 exhibit the benefits of the incorporation of self-healing functionality into a protective coating in comparison to a traditional coating. When a traditional coating is damaged, the underlying substrate is exposed (Figure 2a) and quickly corrode (Fig 2b). The corrosion propagates at the coating-substrate interface, leading to further delamination of the coating (Fig 2c). Damage to a similar coating incorporating self-healing functionality ruptures the incorporated microcapsules, releasing the healing agent into the site of damage (Figure 3a). Once polymerized in the site of damage (Fig 3b), the healing agent seals off the edge of the damage. Although the exposed area corrodes in the case of larger-scale damage, the coating system maintains its adhesion for much longer relative to the traditional coating, while significantly reducing the undercutting.
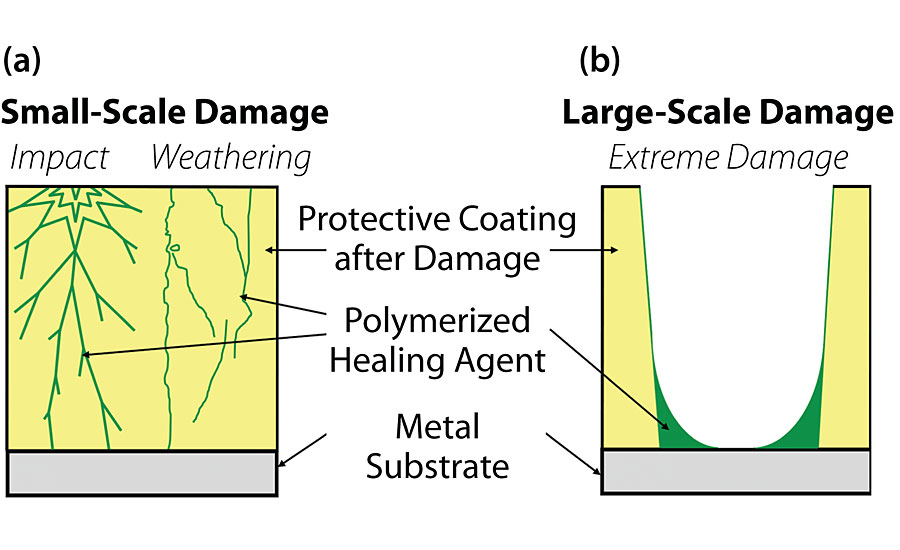
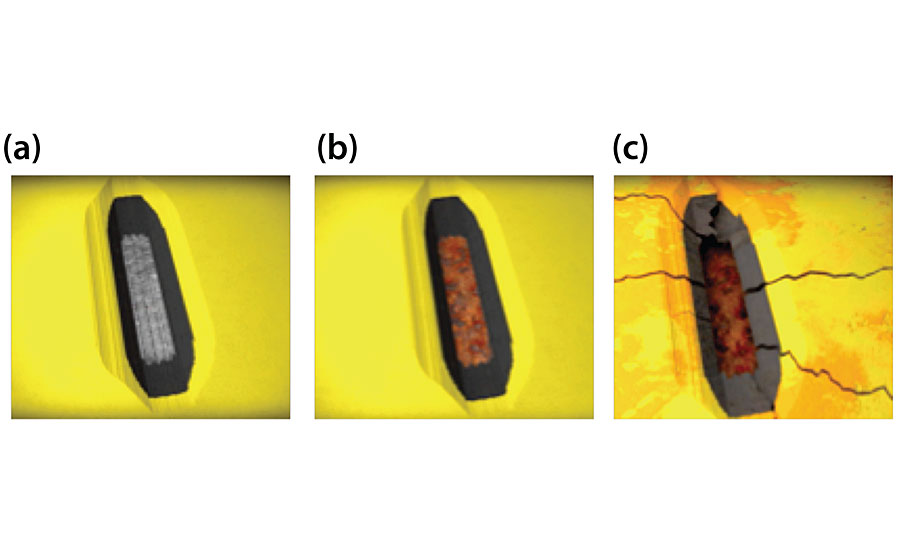
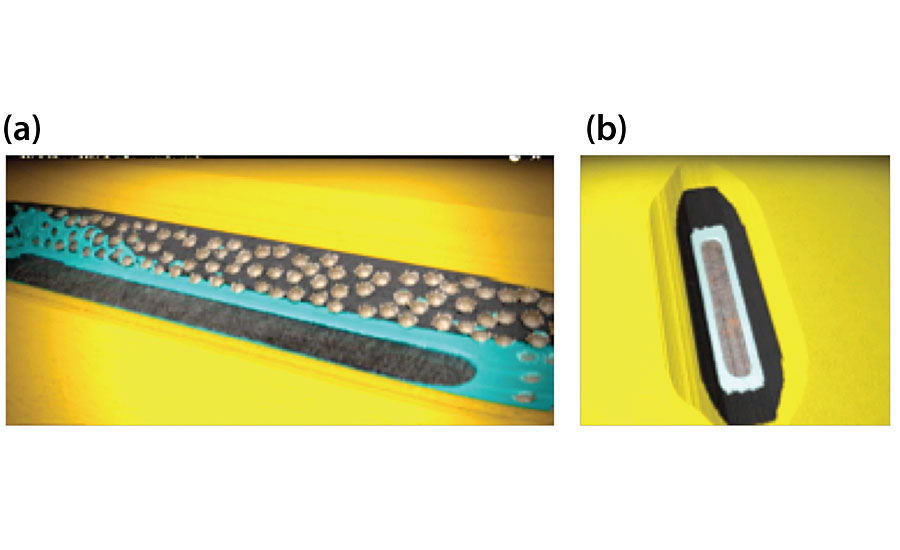
Formulation Optimization and Initial Testing
In order to create METAPrime, a self-healing, high-performance coating, Rust-Oleum modified an epoxy coating and adjusted the formulation to incorporate the self-healing additive. The formulation selected was a two-part polyamide-cured epoxy coating formulated with a base to curing agent ratio of 1:1. A range of self-healing additives at various loadings were first screened using the polyamide-cured epoxy coating as a starting point formulation. The resulting test formulations were applied to cold-rolled steel substrates in two coats with a combined 200 μm (8 mils) DFT, which was at the top of the recommended dry film thickness (DFT) range. Two coats at this target film thickness builds in the best performance advantage by creating a greater loading of self-healing additive in the dry film. Following down-selection of the best performing self-healing additive and loading, a new optimal formulation was designed based on the same resin/curing agent package. Consideration of particle size and oil absorption were important for successful incorporation of the additive into the formulation. The objectives of the product design in addition to the self-healing functionality included the following: (1) maintaining the mechanical properties of the coating, (2) maintaining applicability via spray, brush and roller, (3) maintaining the base/curing agent ratio at 1:1, and (4) minimizing gloss loss to facilitate performance as a universal primer/intermediate coat for a broad range of top coats. Formulation adjustments were also studied to optimize incorporation of the self-healing additive upon production scale up.
The resulting formulation was then tested on cold-rolled steel (CRS) substrates with five disparate top coats including acrylic, alkyd, acrylic-urethane, polyester-urethane and epoxy chemistries. A schematic showing the systems compared is shown in Figure 4. After allowing a minimum of seven days for curing following application of the top coats, two parallel scribes with no less than two inches between them, were made on the coated substrates using a 500-micron scribe tool. The resulting samples were then allowed to equilibrate at room temperature for 24 hrs prior to starting salt fog exposure (ASTM B117). A representative pair of images showing the performance of the self-healing coating relative to the standard analogue is shown in Figure 5a, and a summary of the average coating adhesion loss from the scribe after 1,000 hrs of salt fog exposure for each of the five systems evaluated is shown in Fig 5b. Compared to the control systems, the systems incorporating the self-healing functionality exhibited a minimum of a 50% improvement in adhesion around the scribed areas. The result of the self-healing functionality incorporated into the coating is consistent with the proposed healing mechanism for larger-scale damage shown in Fig 2.
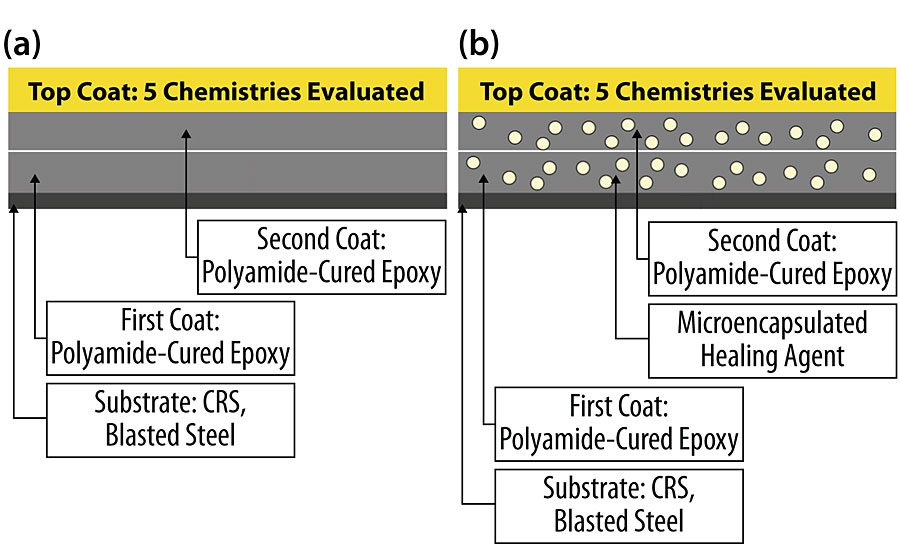
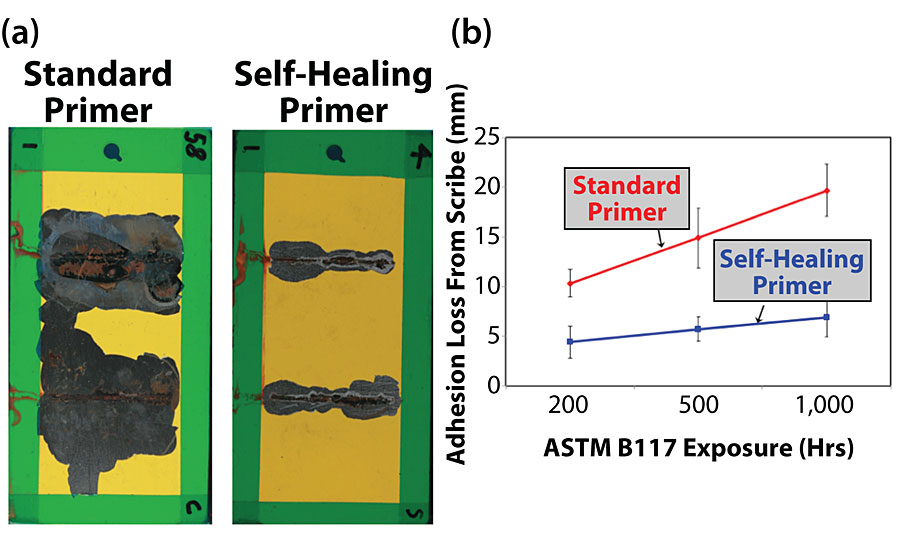
Characterization of the Healing Mechanism
To validate the self-healing technology in a protective coating system in extremely corrosive environments, Chevron and Charter Coating Services (2000) Ltd. designed a test protocol using different analytical methods to further elucidate the healing mechanism of the system. In these experiments, for the control and self-healing systems, abrasive blasted steel substrates were coated with two coats of the epoxy at roughly 100 μm (4 mils) DFT each, followed by a polyurethane top coat applied at roughly 50 μm (2 mils) DFT. A comparison of the cross-sections of both systems via optical microscopy is shown in Figure 6. Microcapsules dispersed throughout the cross-section of the epoxy layers are evident in the image showing the self-healing epoxy coating (Fig 6b). To simulate smaller-scale damage in the coating system such as micro-cracking, a commercially available double-edged blade with a sharp tip and a thickness of about 635 μm (0.025 in) was used to damage the coating system by scribing each coated sample down to the underlying substrate. The samples were then immediately exposed to a salt fog. The samples were evaluated daily to photographically document the kinetics of corrosion on the substrate. The scribe defects on the test samples were also characterized using Optical Profilometry, Scanning Electron Microscopy (SEM)/Energy Dispersive Spectroscopy (EDX) and Elemental Mapping. The results obtained after three days of salt fog exposure are summarized below.
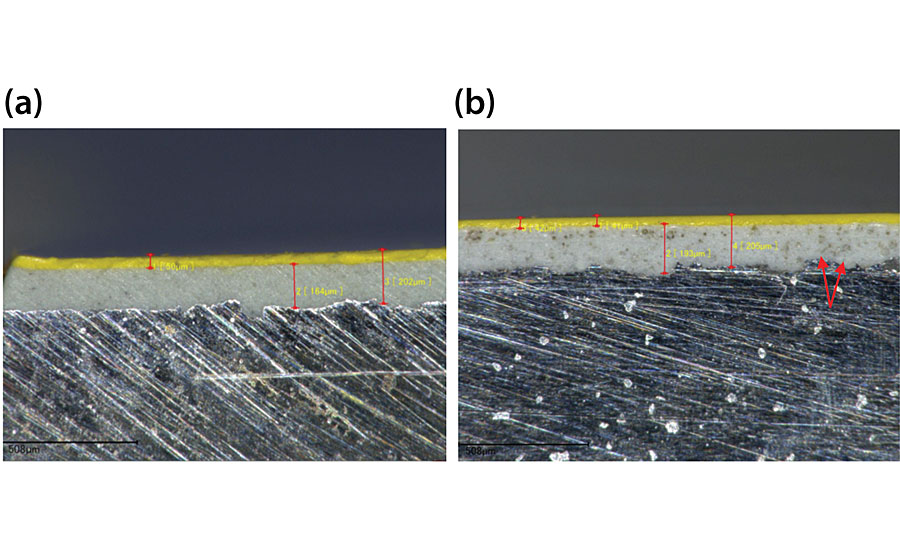
In the case of the control, the initial width and depth at the area selected for analysis were 91 μm and 129 μm respectively (Figures 7a and 7c). The formation of corrosion products was evident within the scribe after 1 day of salt fog exposure. After three days of salt fog exposure, the scribe width was observed to decrease by 40% to 55 μm (Figure 7b), while the depth was observed to decrease to 12 μm (Fig 7d). The change in the scribe width was likely due to some movement by the flexible epoxy resin binder while exposed to the slightly elevated temperature (35C) of the salt fog. The change in the scribe depth for the control sample appears to be largely due to corrosion products forming within the scribe. EDX analysis of the scribe immediately after damage exhibited a strong iron (Fe) signal resulting from the exposed substrate (Fig 7g) and corrosion products could be observed in the scribe by scanning electron microscopy (Fig 7f).
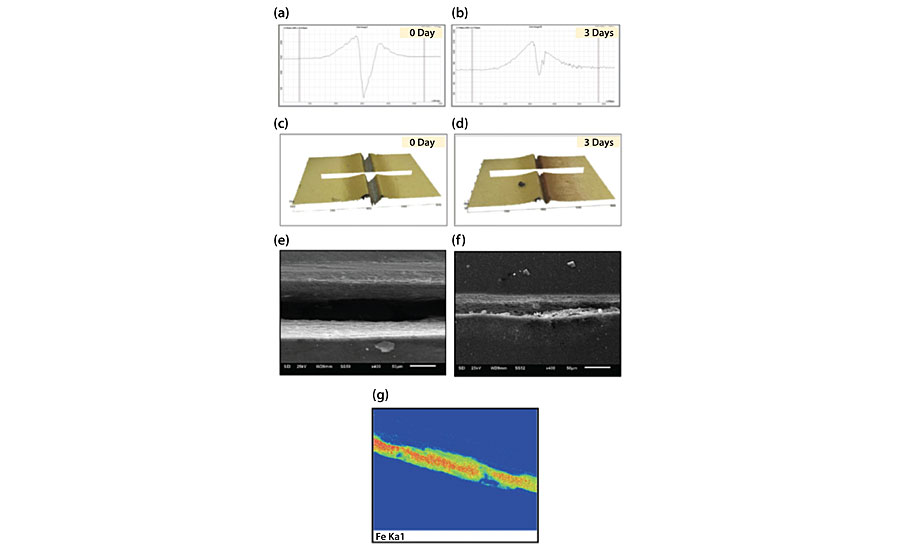
In the case of the self-healing coating system, the initial width and depth at the areas evaluated were 124 μm and 144 μm respectively (Figures 8a and 8c on the following page). No formation of corrosion products within the scribe was evident after 1 day of salt fog exposure. After three days of salt fog exposure, some iron oxide staining of the coating along the scribe line was observed. The scribe width was observed to decrease by 54% to 57 μm, while the depth was observed to decrease by 98% to 3 μm (Figure 8a-d). It should be noted that the changes in the scribe dimensions are significantly greater relative to the control (compare Figures 7a-d to 8a-d). Furthermore, although EDX analysis of the scribe immediately after damage exhibited a strong Fe signal resulting from the exposed substrate (Fig 8g), after three days of salt fog exposure, SEM analysis showed a distinct morphology change within the scribe that was inconsistent with the morphology characterized as corrosion in the control (compare 8f to 7f). EDX and elemental mapping analysis showed no significant deposition or formation of corrosion products within the scribe (Fig 8h). Instead, a uniform distribution of salt deposit was observed on one edge of scribe, likely trapped by polymerized healing agent, preventing it from reaching the substrate (Fig 8i).
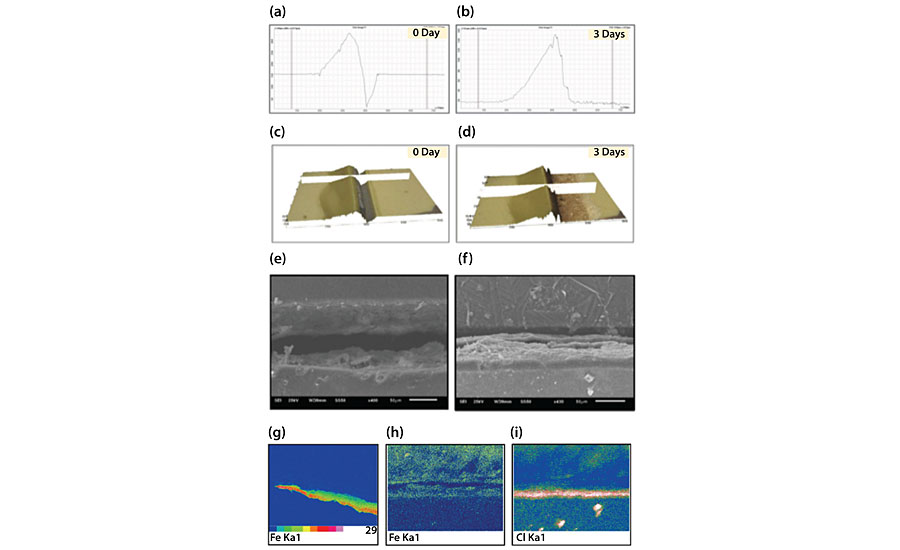
Technology Applications and Value
There is a substantial benefit to extending the life of coatings for offshore and onshore applications. In a recent report, NACE assessed the global annual cost of corrosion to be $US 2.5 trillion, which is equivalent to 3.4% of the global GDP (2013). 10 The study further estimated that savings of up to 35% could be realized on the actual costs of corrosion-related maintenance (not including lost revenue due to downtime) over the life of an asset by simply implementing available corrosion control methods. NACE has also estimated the total cost of marine corrosion worldwide to be between $50-80 billion every year. A separate analysis based on data published in a previously referenced NACE International article 9 has shown that increasing the paint service life by as little 25% (e.g. from 12 years to 15 years) could lead to a cost savings of 32.7%. 11 Note that the preliminary data reported here (Fig 5), suggests the potential of a far greater increase in service life as the performance of the self-healing coating after 1,000 hrs of salt fog exposure exceeds that of the control at 250 hrs. In addition to offshore applications, onshore assets exposed to harsh corrosive environments can also benefit from extending coating life. Although the cost to send maintenance crews to onshore sites is less drastic than offshore, there are still cost savings to be had with the reduction of coating upkeep necessitated by corrosion.
Technology Validation and Conclusions
Our investigation has proven that after inflicting scribe damage to the coatings, the self-healing system releases a healing agent that forms a barrier at the site of damage, resulting in depth reduction of the scribe that significantly surpasses the damage response of the control system (compare Figures 8a and 8b to 7a and 7b).
In addition to validation from the scribe depth profilometry, the high concentration of chloride ions observed on one edge of the scribe (Fig 8i), but notably not on the substrate, is further evidence of the formation of a protective barrier, which appears to have trapped salt from the salt fog in the cured healing agent. Although the self-healing process occurred within the first three days of salt fog exposure, it resulted in a maintenance of adhesion of the coating to the substrate after damage and germane protection of the substrate that led to a 65% decrease in the amount of corrosion creep observed after 1,000 hrs of ASTM B117 exposure (Fig 5b).
The focus of ongoing studies is to assess the robustness of this coating system by conducting a range of cyclic aging resistance tests specified by the oil and gas industry for offshore topsides qualification.
Overall, the research conducted by Chevron, Rust-Oleum, Autonomic Materials and Charter discussed in this article suggests that self-healing additives can be used to improve the performance of a coating system that is subject to mechanical damage, micro-cracking and subsequent corrosion of the substrate while in service. While future work will further develop our understanding of the limitations and functionality afforded by the incorporation of self-healing additives into offshore protective coatings, what we have accomplished so far suggests that coatings exhibiting this kind of damage repair can significantly reduce maintenance costs by optimizing service life and reducing asset downtime.
Note: A version of this article was first published in the May 2020 issue of Materials Performance magazine.
References
1 Blaiszik, B.J.; Kramer, S.L.B.; Olugebefola, S.C.; Moore, J.S.; Sottos, N.R.; White, S.R. “Self-Healing Polymers and Composites,” Annual Review of Materials Research, 2010, 40, 179-211.
2 Wilson, G.O.; Andersson, H.M.; White, S.R.; Sottos, N.R.; Moore, J.S.; Braun, P.V. “Self-Healing Polymers,” Encyclopedia of Polymer Science and Technology, John Wiley & Sons, Inc., 2010, 1-33.
3 White, S.R.; Sottos, N.R.; Geubelle, P.H.; Moore, J.S.; Kessler, M.R.; Sriram, S.R.; Brown, E.N.; Viswanathan, S. “Autonomic Healing of Polymer Composites,” Nature, 2001, 408, 794-797.
4 Cho, S.; White, S.R.; Braun, P.V. “Self-Healing Polymer Coatings” Advanced Materials, 2009, 21, 645 – 649.
5 Wilson, G.O.; Andersson, H.M. “Self-Healing Systems for High-Performance Coatings,” Paint and Coatings Industry, May, 2012.
6 , G.O.; Ebbert, B.R.; Andersson, H.M. “Improved Corrosion Resistance in Powder Coatings via Microencapsulated Self-Healing Agents,” Paint and Coatings Industry, March, 2017.
7 Balijepalli, S.; Nanjundiah, K.; Li, Y.; Barsacchi, M. “Self-Healing Coatings – New Technology Develpments,” Proceedings of the European Coatings Conference, Nuremberg, Germany, April 2009.
8 Ghosh, B.; Urban, M.W. Science, 323, 1458-1460 (2009).
9 Helsel, J.L.; Lanterman, R. “Expected Service Life and Cost Considerations for Maintenance and New Construction Protective Coating Work,” NACE International Corrosion 2016 Conference and Expo, Paper No. 7422.
10 Koch, G.; Varney, J.; Thompson, N.; Moghissi, O.; Gould, M.; Payer, J. “International Measures of Prevention, Application, and Economics of Corrosion Technologies Study,” NACE International Impact, March 2016.
11 Fischer, G; Andersson, H.M. “A New Standard in Corrosion Prevention: How Self-Healing Coatings Enable ROI to End-Users,” NACE Materials Performance Online Platform, http://www.materialsperformance.com/white-papers, Accessed March 31, 2020.
Report Abusive Comment